Everything has its price. Every form of power production brings with it costs in terms of dollars, time, land, materials, pollutants, greenhouse gas emissions, and human deaths.
Here we look at what we have found to be the most important factors for analyzing the feasibility of a proposed power project.
The purpose of this publication is to illustrate the fact that there are a number of important criteria for evaluating the relative advantages and disadvantages of different power sources. Any analysis that proceeds based on only one or a few of these criteria will be incomplete.
Contents
Harmful emissions
Radiation
The two forms of power associated with the largest radioactive emissions are coal and nuclear power. Some people may be surprised to know that more radioactivity is delivered to the environment (and the public) by coal power than from an equivalent amount of nuclear power. We have dealt with this issue in much more detail in our post about the radiation emissions of nuclear and coal power, which is part of our nuclear myth and fact project.
Air pollutants
A number of power sources create a lot of air pollution. Coal power is the most well known of these. We went into great detail about the air pollution of coal in our article coal power: pollution, politics, and profits.
While coal power is the most notorious in terms of air pollutants, other power sources produce some of them too. Nuclear power plants, natural gas turbines, biomass plants, and fuel oil plants all produce some air pollutants. By comparison, renewable forms such as wind, solar, hydro, and tidal produce minuscule amounts of air pollutants.
It is also worth noting that hydroelectric reservoirs, when flooding vegetated land, can trigger the long term release of relatively large amounts of methane as the submerged biomass biodegrades. Methane is a potent greenhouse gas and certainly an air pollutant.
C02 per unit energy
CO2 is an air pollutant that deserves its own section due to its importance. C02 is the greenhouse gas that is the primary force behind human-made climate change. We won’t get into the climate change debate in this article. For a very nice image of what ‘the debate’ is, check out this excellent summary at Information is Beautiful.
C02 emissions per unit energy are now an important factor.
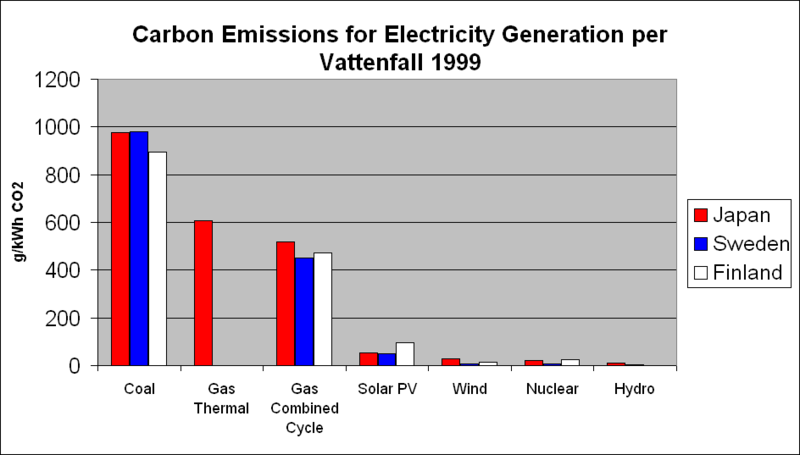
Wastewater and effluents
Effluent is a term referring to water that is returned to lakes and rivers after being contaminated in an industrial process. With regards to power production, the major problems are mostly with coal power effluents, although some of the other power sources such as nuclear may contaminate water to some extent.
Noise pollution
Even turbines on their own make noise. Every power system has a different noise profile. The biggest power plants in the world are generally quite far from residential areas because they can create intense noise pollution. This can apply to thermal plants such as coal, natural gas, and nuclear, but it can also apply to hydroelectricity since the release of water through the dam can be thunderous.
Run of river hydro systems may make some noise, and wind power make some noise. The noise levels of wind power are the subject of some public debate currently, but that is primarily because they pose so little danger in all other ways that people are interested in placing them in or near residential areas.
Comparisons are often made between a wind turbine that is several hundred meters away and a refrigerator heard from another room. In a quantitative sense, the decibel readings of wind turbines that are more than 1 kilometer away are quite small compared with many everyday sounds.
As of yet there are no confirmed health issues from wind turbine sound, but there is certainly a lot of public backlash on the issue. Entire wind projects are being stopped in places such as the UK and US. One of the common topics is the health effects of low-level noise from wind turbines, despite the fact that no such effect has been scientifically confirmed. A 2009 review of the noise pollution from wind turbines found so miniscule of effects that governments around the world are uninterested in researching the matter further because there is no significant threat to their people.1 On the other hand, it seems that the Japanese Environment Ministry will be conducting a review of the sound emission from all of the country’s wind turbines.
The only noise that comes from solar photovoltaic systems would be the rotation motors (if the PV system has systems that angle the panels towards the sun), and the transformers to convert the power to alternating current.
Human lives
All of the above emissions can degrade the longevity and quality-of-life of humans. These are certainly factors that have to be included in any serious consideration of power sources.
However, there are also more acute dangers associated with power sources. Many of these are occupational effects, meaning that they primarily affect workers in the power plants, mines, and supporting industries. Heavy industry can be dangerous, and estimates of the human lives lost in various industries can be very sobering.
There are also acute dangers to members of the public. Hydroelectric dams can fail, flooding land, destroying property, and causing human deaths. Nuclear power plants are famous for the accident at Chernobyl. Natural gas lines leak and explode, often killing civilians, even to this day.2 Coal power air emissions, effluents, and fly-ash spills poison large areas and are considered responsible for large numbers of public deaths.3 See another article of ours that takes a deeper look at the broad issues that contribute to the death toll of coal.
A brief literature review on the Internet found a few studies available online regarding the relative cost in human lives of various electricity generation techniques.
The first article we will mention was published by the World Nuclear Association. While they do seem to have a vested interest in making nuclear look good compared to other sources, they do have some estimates that closely agree with other studies. The full piece is available online as Environment, Health and Safety in Electricity Generation.
Next we will look at a study prepared in 2005 for the Canadian province of Ontario with regards to the economic feasibility of replacing their coal-fired electricity production. The piece makes a strong case against business-as-usual coal power use, since it seems to be the power source that causes the most human deaths per unit energy. It is interesting to note that since the creation of this report, Ontario has directed its efforts heavily towards renewable energy through feed in tariffs.
Lastly, we found this report on various options for electricity production in various European Union countries. Some very interesting conclusions are drawn from this analysis that are worth noting. There are some very clear answers to questions regarding the societal health effects of some forms of electrical power generation. For example, hydro and nuclear power are two orders of magnitude less of a risk than coal and oil. For those that are new to the term, and order of magnitude is a power of ten, so two orders of magnitude less risk means approximately 100 times less risk.
An interesting extension of this reasoning can be added in the case of oil and other contested resources. It is well understood that oil has been a valuable enough energy resource that wars have been fought, and continue to be fought, over its acquisition. Adding in the deaths, stresses, expenses, and damage to infrastructure from oil wars would raise its already notoriously high detrimental human health effects.
Land
Areal power density
We looked into the issue of areal power density in some detail in a previous issue of the Renewable Energy Review titled: Land use of coal vs wind: Still room for debate. In that publication we show how to perform simple calculations of areal power density, and show some detailed examples.
People familiar with the work of Vaclav Smil will know this criteria as ‘power density’. One of the only criticisms that we level at Smil’s work is that he tends to overstate the significance of power density when considering possible future plans for our society’s energy future. We acknowledge that power density is a very important factor to consider, but disagree about the extent to which it is weighted against all of the other criteria listed in this article. Additionally, we did comment on what we identified as some notable methodology gaps in his analysis of coal and wind power in our look at the land use of coal vs wind. We will now discuss those issues briefly here.
Coal power
Coal power has a growing areal footprint. It damages incrementally more land with mining and fly ash storage. It also damages arable land with airborne toxins and water effluents. Also, new coal development is taking place with lower quality coal seams and less-than-ideal locations that may be further from mining locations, cooling water, and population centers. Finally, if we add in the dangers of land damage and flooding due to climate change and sea level rise caused by the C02 emissions from coal, there will also be some substantial changes to the relatively high power density of coal.
Wind power
As for wind power, we feel that great care must be taken in assigning an areal power density number. Firstly, the land used for wind power can also be used for other things such as herding and farming. It is not an exclusive use of land like a coal plant or mining location is. Secondly, existing wind power installations, which Smil uses for his analysis, are not optimized to maximize power density. They are optimized for cost-effectiveness.
Today, turbines are typically much more expensive than the land that they will be placed on. This means that in order to optimize costs, the turbines are placed rather far from each other to minimize their interference with one another. By looking at the areal power density of existing wind farms, we are not looking at how high the power density can be. We are merely getting a snapshot of what the power density of wind is when it is optimized for costs in the current turbine and land markets. As the best locations for wind are filled with turbines, and if land becomes more expensive, we are likely to see some notable increases in the areal power density of wind.
Lastly, wind power lacks the incrementally growing land use of coal. The land formerly occupied by wind power turbines is unharmed and able to be used after the wind power systems have been taken down.
Solar synergies
There are some other land use synergies for solar photovoltaics. They can be placed on building roofs. Even considering only the south-facing roofs without obstructions in the northern hemisphere, this is a very substantial area that is already in use. Also, it is possible to use a short herding animal such as sheep to keep the grass short in fields of photovoltaics. There has been an unfortunate tendency in some places to sterilize the ground under photovoltaic installations so that nothing grows there. We believe that grazing animals would be a better solution.
Siting requirements
Certain types of power plants can only be built in certain places. For example, hydroelectricity needs a lake, river or stream. Some other power plants are more effective in certain places, but can be built almost anywhere. For example, solar power works best with maximal direct sunlight, so building in an area permanently shadowed by a mountain for instance would be a really bad idea, but it can be done. Solar panels can produce some energy even from diffuse light.
Thermal power plants such as coal, natural gas, and nuclear produce about 80% of the world’s electricity. These systems require both a heat source such as coal, natural gas, or nuclear energy, but they also require a way to cool themselves. This is because heat engines operate based on differences in temperatures. Generally the cooling is accomplished using a large body of water, a river, or cooling towers. The most cost-effective thermal plants are generally near large sources of water that they can use for cooling.
Money
The issue of capital costs tends to dominate our discussion of many issues, including power production. What are the facts that we consider then regarding the relative costs of power generation equipment?
Construction time
It is important to keep in mind that an important factor in the cost-effectiveness of a power plant is the length of time it takes to design, certify, and build. Nuclear power plants have become notorious for coming in substantially over budget. Much of these cost increases are actually due to interest accruing on the several billion dollar loan that is taken out to fund the construction of a new nuclear plant. Delays of months can be expensive. Delays of years can be debilitating.
Changes in governmental regulation have sometimes been the culprits for delays in nuclear plant construction. There has also been a failure to utilize modular designs with quick construction times. To some extent this may be excusable because of the public safety role of regulation, but we believe that a lot of improvement is possible.
Realistic costs for all long term projects should include provisions for unexpected delays. However, we have only run into a few power cost estimates that take into account these delays. Most cost estimations for future development are thus typically a bit low. We feel that this is misleading with regards to power plants that take a long time to build, such as nuclear power, because even slight delays (which are common) will introduce very notable additional expense.
Cost per installed kilowatt
Here we are talking about how much money it costs to create a power plant per kilowatt of generating power. If you are unfamiliar with the term kilowatt or kilowatt-hour, see our piece on energy definitions.
For example, in March 2009 I was quoted a price of $13,000 for a grid-tie photovoltaic system that had a max power of 1.9 kilowatts. What would our cost per installed kilowatt be for this system? We take $13,000 and divide it by 1.9 to get $6842 per installed kilowatt. People familiar with power systems may see that this is a relatively high cost. This is partially due to the fact that power systems have large economies of scale. It is also due to the fact that solar photovoltaics are reasonably expensive for personal use.
The local utility estimated their costs for creating various forms of power production in their publication: SaskPower: Powering the future. They cite for instance the cost per installed kilowatt for large-scale wind power to be $2000-$3000, for solar photovoltaics between $7,000 to $13,000, and for large-scale ‘compliant’ coal about $4,300 to $5,700. They also cite prices for natural gas, hydro, and biomass which we will not repeat here. These prices are ‘overnight’ costs, where the plant is theoretically built instantly. These costs do not include interest charges on debts, inflation, or escalating labour costs. All of these factors would be expected to increase these prices by some amount.
Cost per kilowatt-hour
While a kilowatt is a unit of power, a kilowatt-hour is a unit of energy. For those of you who are not as familiar with these units, it may be helpful to look at this brief explanation of power vs energy at Wikipedia.
Here we are discussing the cost of energy produced by a power system, rather than its maximal power rating. In order to understand this concept deeply, it must be understood that all power systems have a capacity factor. The capacity factor is the fraction of the amount of energy actually produced over the amount of energy that could possibly have been produced if the power system had been producing maximally.
For example, a home wind turbine might have a power rating of 1 kilowatt. However, over the course of 10 hours it might only have produced 2 kilowatt-hours because the wind was not going fast enough for it to produce at the peak rate. The turbine had a theoretical maximum energy potential during those 10 hours of 10 kilowatt-hours (power of 1 kilowatt multiplied by the number of hours it was running). If we calculate 2 kwh / 10 kwh we get 0.20 or 20%. We would say that for this 10 hour segment this wind turbine had a capacity factor of 20%.
Cost per kilowatt-hour refers to the monetary cost of a kilowatt-hour of energy from a particular power source. This is definitely one of the most important economic factors for a power system. Why? Because power plants are generally paid primarily based on how much total energy they produce. At least two distinct caveats must be immediately applied to this statement. There are at least two major factors that will change the price being paid for a unit of electric energy: 1) Whether the power is dispatchable, or there-when-you-ask-for-it, which we look at later on in this article. 2) Whether the power is available near peak-demand times such as mid-afternoon on a hot summer day in California. During these times electricity is being demanded much more than at other times.
With the above caveats in mind, we can still state with confidence that cost per kilowatt-hour of electricity is the major driving force for the economic viability of power systems. A good example of this would be wind power. Wind power has seen incredible growth in the world in the last decade despite the fact that it is neither dispatchable or predictably available at times of peak demand. Its economic viability is based almost entirely on its relatively low cost per kilowatt-hour of electricity. That is, wind might not produce the power when you need it, but it can produce cost-effective power during its lifetime.
Since this is the most important economic metric for power systems, there has been a lot of research about the comparative costs of various forms of power. We will not recite these studies here, but we can point out the best ones that we are aware of.
A nice review article on the subject is the Wikipedia article on Cost of electricity by source. In it, they go through the general methodologies for such an analysis, and then summarize the findings of several major studies of costs. The broadest study seems to be that one completed by the U.S. Department of Energy, which is summarized below:
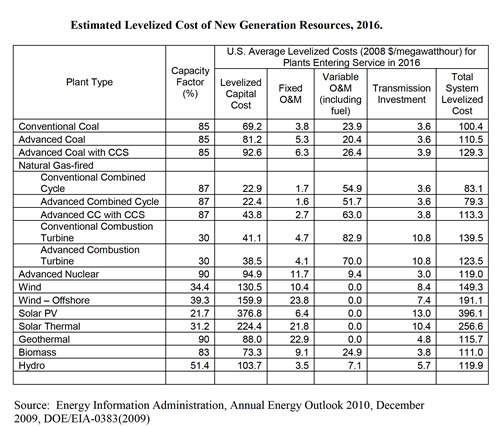
When reading the summary tables, the costs are often given in dollars per megawatt-hour (MWh). A megawatt-hour is one thousand kilowatt-hours. The price of electricity that is paid by a consumer for their home in North America is usually around $0.10 per kilowatt-hour, which works out to $100 per MWh. Somewhat similar rates can be found around the world.
We feel that it is important to point out that tables of costs per installed watt are very location specific. Different geographic regions have different labour costs, specialized industries, land costs, and natural resources. Natural resources in this case refers to everything from domestic natural gas to rivers, wind and sunlight. An obvious example would be that Nevada and Spain get a lot more sun than Alaska.
All of the major studies in the Wikipedia article include some degree of geographic specificity. This is necessary in order for these studies to be meaningful. There is no unified cost estimate for everywhere in the world. Building things in different places costs different amounts of worker time as well as physical resources. As pointed out above, the energy resources of different locations can also be quite different.
Energy characteristics
There are a number of important factors to consider when examining the abilities of power generation equipment.
Energy return on investment
Energy return on energy invested (EROEI) is the ratio of the amount of electricity produced by a piece of equipment over the amount of energy required to create that piece of equipment. For example, if during its entire lifetime a hydroelectric turbine created 100 MWh of energy and it took 2 MWh to build the turbine, then the EROEI of this turbine would be 50.
EROEI is important because it puts some fundamental limits on how quickly we can add power generation to our grid. For example, our above example of the hydro turbine should be able to, during its lifetime, pay back the energy debt for its own construction and then a surplus of 98MWh. This could in theory provide enough energy for the creation of 49 more turbines like it. This number is rather high. Most fossil fuel and nuclear power systems that we are aware of have smaller EROEIs in the range of 4 to 20.
Fossil fuel EROEI tends to fall
EROEI tends to fall over time for non-renewable resources such as oil, coal, and natural gas. This is because the most easily accessible resources are generally exploited first. Accessibility is comprised of a combination of location, difficulty in extraction, and difficulty in refinement. For instance, oil in Saudi Arabia is often cited as having an EROEI of approximately ten. This can be compared to oil is North America which we have seen cited as having an EROEI of approximately five!4 It is clear that technological improvements in the extraction, transport, and refining of fossil fuels have not outpaced the increased difficulty of more remote and lower-quality resources. These decreasing EROEIs for fossil fuels have definite consequences for the energy fate of our civilization.
Farmland
Farmland has also seen decreasing EROEIs in recent decades. This may sound strange since our food yields have been increasing. The fact is however, that we are using much larger amounts of energy to work our land and to create and distribute the growing amounts of pesticides, herbicides, and fertilizers. Many of the chemical bases of these products are drawn from non-renewable resources. For instance, potash (a non-renewable resource) is being heavily mined to produce fertilizer through the very energy-intensive Haber-Bosch process. Our farming practices today are relying heavily on huge energy and resource inputs from elsewhere. It is clear that farming practices will need to be refined in the coming decades or face skyrocketing EROEIs. The alternative is to use even more energy to create these additive chemicals. This additional energy might be used to mine increasingly remote and low-quality deposits of raw materials, or perhaps to create fertilizer through energy-expensive techniques.
Solar is rising
On the other hand, EROEIs for renewable energy sources are on the rise. Thin film photovoltaics have recently been cited at 30, and some even above that high value.5 Concentrated solar thermal power has been cited as having EROEIs between 30 and 70.6
Wind and hydro very high
The Encyclopedia of Earth’s article on the Energy Return on Energy Invested for a number of existing wind turbine installations demonstrates an average result of 19.8 for the EROEI of wind power. This is a number they claim is quite high when compared to conventional power sources (other than hydro). Some individual turbines were in the single digits, while some others were as high as 30 to 70. It is also worth noting that all but one of the turbines studied were analysed through publications from before the year 2002. Wind power has made great progress since then, and we would expect that its EROEIs have improved by some amount in the years since.
Drawing upon the links and references given above, it is clear that the EROEI of hydro power also tends to be very impressive, in the range of 20 to 200. There is a reason why hydro is still the king of renewable energy in terms of power produced. Hydro is an incredible resource, but care must be taken with further development because we have used many of the ideal locations already.
Energy payback time
Energy payback time is closely related to the EROEIs that we just looked at. Energy payback time refers to how long a piece of power generation equipment needs to be in operation before it creates the same amount of usable electricity as the amount of energy that went into constructing it.
We can calculate energy payback time from the EROEI if we know the average lifetime of the power plants used to create the EROEI estimate. We would take the lifetime and divide it by the EROEI to get an estimate of the energy payback time.
For example, an article at JGZ estimates of the energy payback time of concentrated solar thermal power is around 8 months, a very impressive number! Energy pay back times for photovoltaics are estimated by the National Renewable Energy Laboratory in the United States to be between 1 and 3.8 years, depending on the technology and implementation.7 Again, these are very impressive numbers.
An earlier citation from Real Energy gives some useful numbers for the calculation of energy payback times from EROEIs. It is clear that most of the conventional fossil fuel sources have relatively high energy payback times while the currently developing renewables are quite impressive.
It is important to note that EROEI and energy payback time numbers are not the same for every installation for a given type of power. Techniques vary, as do the quality of resources such as wind and hydro. There is room for innovation and improvement for all energy types. Our understanding of physics leads us to the belief that the conventional fossil fuels are not going to substantially improve their EROEIs or energy payback times with new innovations. We are rapidly reaching the limits of what those energy resources can do for us.
On the other hand, there are still very impressive gains expected for the renewable energy forms. Most of them are young industries that are currently driving innovation at a spectacular rate. Another notable exception may be the development of advanced nuclear reactors. Reactors such as the Liquid Fluoride Thorium Reactor (LFTR) may be incredible power sources, if they are researched and developed. There is currently an ongoing project at Energy from Thorium to encourage investment in the development of LFTR. To that end, Energy from Thorium has collected all the relevant science and scholarship that they can get their hands on. They make an impressive case for LFTR, convincing us that it certainly deserves to be researched.
Lifetime
The lifetime of a proposed power plant is important for a number of reasons. Long-term planning by power authorities will examine the entire lifecycle of the power resource. The lifetime of a power system will also affect planning by local communities and investors.
Dispatchability
We looked in great detail at the concept of dispatchable power in a previous publication of the Renewable Energy Review. Essentially if a power system is ‘dispatchable’, it means that we can turn it on and off at will in seconds, minutes, or hours. If a power system takes most of a day to turn on, it is likely to be considered a baseload source rather than a dispatchable one.
Intermittency
Power sources that are intermittent are power sources that are not baseload or dispatchable. These are sources such as wind power and solar photovoltaics that only produce power when there is wind or sunshine respectively.
In the case of wind power, it is worth mentioning another facet of its limitations. Some areas of the world experience temperatures below -20ºC. In these places, wind turbines often have an automatic shutdown temperature at around -30ºC. This is not always the case however, since there are functioning wind turbines on Antarctica where it gets much colder.
However, the fact remains that many wind power installations will not produce power when it is extremely cold. This is problematic for my home province of Saskatchewan since the maximum demand is usually during the coldest times of the year.
Predictability
The predictability of an intermittent power source is very important for grid control operations. In order to ensure grid stability, we need to be able to match the drops in intermittent power production with dispatchable sources.
Energy resources can vary a lot in terms of how predictable they are. For example, in our home province of Saskatchewan, the wind is often predictable on a scale of hours to even days. Run-of-river hydroelectricity in our neighboring province of Manitoba is very predictable on the scale of weeks to seasons.
The key point here is that not all intermittent sources have the same character of intermittency. Depending on the resource type (wind, solar, hydro, etc) and the specific location, predictability can vary greatly.
With the exception of hydroelectricity, most of the fastest-responding dispatchable sources such as natural gas and energy storage are quite expensive. In general it is true that the grid management costs will decrease with increasing predictability.
Today’s energy market currently favours wind power despite its unpredictable nature. This is because the grid management costs thus far have been relatively small. The low price of wind energy makes it an attractive investment in many places even though it is usually relatively unpredictable.
Load-following
Intermittent power sources can sometimes follow the patterns of usage to some extent. For example, solar photovoltaics produce the most power near the middle of the day, which often coincides with high power use due to things such as air conditioning during hot days. Thus to some extent solar photovoltaics produce power when it is needed most in these areas.
However, many places in the world have a midwinter peak power usage. This is true for our home province of Saskatchewan. The maximum power usage in a Saskatchewan year is usually on or near the coldest days of the year. In this setting solar photovoltaics would be extremely poor at matching the load at all.
Wind power in Saskatchewan has been found to produce more energy during winter. This helps a bit with meeting the energy requirements during these times. However, as we looked at above, most wind power turbines can only function above temperatures of -30ºC.
- Wikipedia: Environmental effects of wind power: 2009 Review. Accessed November 24th, 2010. [↩]
- 2010 San Bruno Pipeline Explosion. Wikipedia. Accessed November 25th, 2010. [↩]
- China is the chief bad example for coal deaths in the world today, as evidenced by this section of the Wikipedia article on coal power in china. Accessed November 25th, 2010. [↩]
- Wikipedia: Energy Returned on Energy Invested. Accessed November 24th, 2010. [↩]
- Real Energy: EROEI. Accessed November 24th, 2010. [↩]
- JGZ: Implications of EROEI ratios. Accessed November 24th, 2010. [↩]
- National Renewable Energy Laboratory. What is the Energy Payback for PV? Accessed November 24th, 2010. [↩]
3 thoughts to “Power system performance metrics”